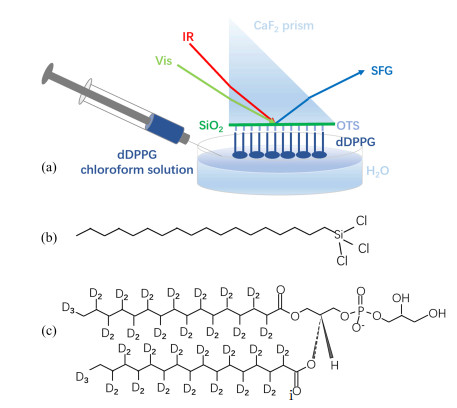
Citation: | Xiao-feng Han, Wen-hua Sun, Shu-jing Wang, Xiao-lin Lu. Sum Frequency Spectroscopy Studies on Cell Membrane Fusion Induced by Divalent Cations[J]. Chinese Journal of Chemical Physics , 2022, 35(6): 883-892. DOI: 10.1063/1674-0068/cjcp2110213 |
Cell membrane fusion refers to the biological phenomenon in which two lipid bilayers contact each other and fuse into an interconnected structure. First, this process is actively involved in a number of vital cell functionalities, such as fertilization [1], mitochondrial fusion [2], and endocytosis [3], etc., thus playing significant roles in the reproduction, growth and ending of the life. Second, numerous diseases lean upon membrane fusion, for example, fusion of host cells and the enveloped pathogens [4, 5]. To this end, this process can be applied for the development of biomedicines [6], e.g., indispensable membrane fusion for delivery of the therapeutically encapsulated antibodies or exogenous genes into the focal cells [7].
As is known, membrane fusion contains a series of intricate physiological events, which have been extensively investigated [8−17]. However, the exact mechanism behind has been poorly understood. Spontaneous membrane fusion is mainly regulated by membrane proteins, such as soluble N-ethylmaleimide-sensitive factor (NSF) attachment protein receptors (SNAREs) [8, 9]. In vitro lipid bilayer fusion can be artificially mediated by polyethylene glycol (PEG), polypeptides, and calcium ions [10−12]. To build the effective investigation model and avoid negative effect from redundant components in cell membranes, model cells, e.g. lipid liposomes, have often been utilized to mimic real cells in order to study the cell membrane fusion. Currently, assays for evaluating membrane fusion are mainly based on concentration dependent effects of fluorescent probes, including NBD-Rhodamine energy transfer [13], pyrene excimer formation [14], and octadecyl Rhodamine B self-quenching [15].
The membrane fusion process is generally divided into four steps [16]. Initially, the involved membranes are about to approach to each other within several nanometers apart. Secondly, dehydration of the phospholipid molecules occurs to break through the repulsive force from the adjacent water molecules bound to the bilayers, therefore the lipid bilayers can further get closer within several angstroms. Afterwards, in the closest contact regions, the destabilized bilayers trigger off rearrangement of the local lipid molecules. Eventually, the corresponding parts will join together and the connected portions will spread outward, ultimately leading to the blending of endocytes [17].
As mentioned above, the cell membrane fusion involves the rearrangement of the phospholipid molecules and dehydration of the water molecules at the bilayer surfaces. To this purpose, a surface/interface sensitive technique, sum frequency generation vibrational spectroscopy (SFG-VS) [18−35] is an ideal tool to study the cell membranes and the related dynamic processes [36−51], including the phospholipid bilayers/monolayers in response to the divalent metal ions [52−55] and the interactions between fusion peptides/proteins and model cell membrane [56, 57].
In this work, we applied SFG-VS to study the membrane fusion of negatively charged lipids induced by divalent cations. Due to the electrostatic attraction, the divalent cations would bind to the negatively charged lipid surface, screen the surface negative charges, diminish the electrostatic repulsion and allow the membranes to approach to each other, finally resulting in the membrane fusion [58]. It should be noted, charge screening is not the only factor for the membrane fusion. The mechanism behind is still not very clear enough. As the same class of homologous ions, Ca2+ and Mg2+ exhibit very different characteristics in the membrane fusion process [59]. The apparent experiment for synthetic phospholipid vesicles demonstrated that Mg2+ can only induce extensive aggregation of the lipid vesicles, but Ca2+ can effectively induce the membrane fusion [59]. In this work, to understand the intrinsic mechanism of the membrane fusion process, SFG-VS and the dynamic light scattering (DLS) were employed to trace the membrane fusion process induced by Ca2+ in comparison with the case using Mg2+. The local structural responses of the model phospholipid monolayers in vesicles and on supported substrates to the two divalent metal ions, e.g. the orientation and dehydration behaviors of the phospholipid molecules, were systematically investigated, revealing the different interaction processes with the two metal ions at the molecular level.
1,2-dioleoyl-sn-glycero-3-phospho-(1′-rac-glycerol) (DOPG), 1,2-dipalmitoyl-sn-glycero-3-phospho-(1-rac-glycerol) (DPPG) powders and the vesicle extruder were purchased from Avanti Polar Lipids. The liposome samples were prepared via the mini-extruder extrusion technique referring to the official website of Avanti Polar Lipids [60]. To be more specific, 8 mg of dry lipid powders was dissolved in trichloromethane in a polycarbonate (PC) test tube. The solvent is removed via nitrogen drying and a lipid film is produced on the tube wall. Place the tube incompletely sealed and placed in a vacuum dryer overnight. A lipid suspension with a concentration of 4 mg/mL was prepared by hydrating the dried lipid film with a 0.01 mol/L phosphate buffer saline (1×PBS, pH=7.0) solution. The lipid suspension was then extruded to obtain the liposome or vesicle solution using the PC membrane with a pore size of 200 nm. Attention should be paid, during the hydration and extrusion processes, the temperature should be kept above 60 ∘C.
In the SFG-VS experiment, a near total internal reflection geometry was adopted (FIG. 1). The supported substrates used for the SFG experiments were the CaF2 prisms. To break the inversion symmetry of the phospholipid samples for the SFG activity, an octadecyltrichlorosilane (FIG. 1(b)) self-assembled monolayer (OTS SAM)-lipid monolayer system was constructed, as shown in FIG. 1(a). First, a 100 nm-thick SiO2 film was deposited on the CaF2 prism substrate by the physical vapor deposition method. An OTS SAM was then deposited on the SiO2 film surface to mimic the inner leaflet of a lipid bilayer (instead of using a lipid monolayer). After that, the OTS SAM was placed to contact with a dDPPG, (1,2-dipalmitoyl-d70-sn-glycero-3-phospho-(1-rac-glycerol), FIG. 1(c)), phospholipid monolayer on the water surface to form an OTS SAM-lipid monolayer system, serving as a model cell membrane.
The theory and background of SFG-VS have been extensively introduced [18−34] and will be not repeated here. SFG-VS has been extensively applied to study the lipid monolayers, bilayers, and related peptides and proteins [35−51, 56, 57]. The SFG spectrometer in this study was customized from EKSPLA. The optical source is a 25 ps pulsed laser with the output wavelength of 1064 nm, repetition frequency of 20 Hz, and energy of 30 mJ. Through the nonlinear optical processes, including frequency doubling, frequency tripling, optical parametric generation/amplification (OPG/OPA) and difference frequency generation (DFG), a 532.1 nm visible beam and a wavelength-tunable mid-infrared (MIR) beam required for SFG-VS were generated. The visible and MIR beams were temporally and spatially overlapped at the OTS SAM-lipid sample interface to generate the SFG vibrational signals. The SFG spectra were obtained by recording the SFG signal intensity as a function of the input MIR wavenumber using a monochromator and a photomultiplier tube (PMT) module.
The SFG spectra in the PO, CO, CD, CH, and OH stretching regions were collected to probe different local molecular information for different functional groups in the OTS-dDPPG bilayer. Three experimental conditions were used for the OTS-dDPPG system (FIG. 2) : (ⅰ) without metal ions: SFG spectra were collected from the interface between the OTS-dDPPG system and water (FIG. 2, left panel). (ⅱ) trans-complexation with metal ions: SFG spectra were collected from the OTS-dDPPG/metal ion solution interface (FIG. 2, middle panel). (ⅲ) cis-complexation with metal ions: the OTS-dDPPG system was placed in contact with the metal ion solution. The DPPG vesicles were then added into the metal ion solution. The volume ratio of the DPPG vesicle solution to metal ion solution was 1:20. The SFG spectra were then collected from the OTS-dDPPG/metal ion solution interface. In this case, the phospholipid molecules were present on both sides of the interfacial metal ions (FIG. 2, right panel). In SFG experiments, deuterated DPPG (dDPPG) was used as the OTS SAM-lipid. In this way, we can measure the CD groups and distinguish them from the CH groups in OTS SAMs. At room temperature, DPPG bilayer is in gel phase, which can effectively avoid the fusion process of vesicles and OTS-DDPPG layer.
The orientation angles of different phospholipid molecular groups at the interface can be quantitatively analyzed [19, 20, 46, 47]. The SFG spectra with different polarization combinations (such as ssp: s-polarized sum frequency signal, s-polarized visible beam, and p-polarized mid-IR beam, corresponding to the effective second order nonlinear susceptibility χ(2)eff,ssp) were first collected. Based on the relationship between the polarization directions of the laser beams (s or p) and the laboratory coordinate (x, y, z) system, after de-convoluting the Fresnel coefficients of the light reflection and transmission at the interface, ratios between the second order nonlinear susceptibility tensors in the laboratory coordinate frame, χ(2)ijk (i, j, and k are the laboratory coordinate axes, for example ssp corresponds to χ(2)yyz for isotropic XY interface) can be calculated from the measured SFG spectra. The hyper-polarizability tensors defined in the molecular coordinate system, β(2)abc (a, b, and c are the molecular coordinate axes), can be connected to χ(2)ijk with the Euler transformation [19, 20]. Through the relationships among the corresponding of χ(2)eff, χ(2)ijk, and β(2)abc, the Euler transformation angles, θ, ψ, φ, which define the orientations of the functional groups at the interface, can be determined. In this study, this method was adopted to deduce the orientation angles of the various phospholipid molecular groups at the interface.
The size distribution of DOPG vesicles was measured by DLS, as shown in FIG. 3. The results show that Ca2+ ions have induced the increase of the average lipid vesicle size from ∼200 nm to ∼600 nm (FIG. 3(a)); while Mg2+ had almost no effect on the lipid vesicle size (FIG. 3(b)). This indicates that Ca2+ can effectively enable the vesicle fusion, but Mg2+ can not, which is consistent with the previous report observed using the electron microscopy [59].
To further explore the mechanism of the Ca2+-induced membrane fusion, different phospholipid sample systems, as shown in FIG. 2, were investigated upon exposure to Ca2+ and Mg2+ using SFG-VS. As mentioned before, the OTS-dDPPG bilayer system with a hydrogenated OTS SAM and a deuterated lipid leaflet was applied to break the inversion symmetry for the SFG activity. The SFG-VS measurements in different vibrational frequency regions were performed to examine the local structural responses upon exposure to Ca2+ and Mg2+.
The SFG spectra contributed by the PO2− groups in the lipid molecules are shown in FIG. 4. Basically, two peaks can be observed from the lipid hydrophilic head groups. The peak centered at ∼1095 cm−1 is contributed by the PO2− symmetric stretching (ss) mode [46, 47, 52] and the peak centered at ∼1070 cm−1 is assigned to the phosphate ester stretching mode (C−O−P) [52]. In the absence of the metal ions, as shown in FIG. 4(a), the peak center of the PO2− group is located at ∼1095 cm−1. With the lipid leaflet in contact with Ca2+ (FIG. 4(b)), a blue shift of the PO2− peak center to ∼1110 cm−1 is observed. Exposure of the lipid leaflet to Mg2+ (FIG. 4(c)) causes a blue shift of the PO2− stretching vibration as well, although the shift is smaller, only 1104 cm−1. It has been reported that the peak center of the PO2− symmetric stretching (ss) mode appears at ∼1100 cm−1 when there exists the hydrogen bonding between PO2− and H2O. So the blue shift of the PO2− ss mode observed here indicates that the binding between PO2− and H2O is weakened due to the competitive interaction with the metal ions, e.g. Ca2+ and Mg2+; meanwhile, the hydrogen bonding between the lipid hydrophilic head groups and water molecules was weakened more upon the metal ion interaction from Ca2+ than that from Mg2+.
As anticipated, the carbonyl groups in the OTS-dDPPG lipid monlayer system have generated strong SFG signals in the CO stretching region from 1700 cm−1 to ∼1750 cm−1. The SFG spectra of the CO stretching mode of the lipid molecules in the OTS-dDPPG monolayer with and without the interaction with the metal ions are shown in FIG. 5. As shown in FIG. 5(a), there are two peaks at ∼1720 cm−1 and ∼1735 cm−1 with the opposite signs (especially in ppp spectrum). This phenomenon is consistent with the previous report by Dreier et al. that there were two distinct carbonyl moieties in the lipid monolayer in contact with water with opposite orientations with respect to the interface plane [52]. According to this, we attribute the low-frequency band at ∼1720 cm−1 to the C=O stretching mode with the oxygen atom pointing toward the water phase with the C=O groups in the hydrophilic environment. Compared to the band at ∼1720 cm−1, the sign of the high-frequency one at ∼1735 cm−1 is opposite, indicating these carbonyl moieties with the oxygen atoms pointing away from the water to the lipid alkyl tails with the C=O groups in the hydrophobic environment. Because the vibrational spectral line shape for the band at ∼1735 cm−1 is highly interfered, as shown in FIG. 5, we only analyze the orientations of the C=O groups with the oxygen atoms pointing toward the water phase through the SFG spectral band at ∼1720 cm−1 upon the interaction with Ca2+ and Mg2+. After fitting the SFG spectra, the ratio of the second order nonlinear susceptibility components χ(2)ppp/χ(2)ssp is measured to be 1.66 for the case of the OTS-dDPPG sample interacting with the water without the metal ions, as shown in FIG. 5(a). FIG. 5 shows the SFG spectra in the C=O stretching region when the phospholipid molecules interact with Ca2+ or Mg2+ with or without the lipid vesicles in contact with the OTS-dDPPG monolayer. We define two situations, i.e., the OTS-dDPPG monolayer interacting with the two metal ions with and without the presence of the lipid vesicles as trans- and cis- ligand bindings, respectively. There is no distinct peak shift of the C=O stretching mode in any of the four cases, indicating that the metal ions do not bind to the C=O groups. The fitted values of χ(2)ppp/χ(2)ssp (∼1720 cm−1) are 0.58, 0.42, 0.66, and 0.56 for the spectra shown in FIG. 5 (b), (c), (d), and (e), respectively, corresponding to the phospholipids trans-complexed with Ca2+, trans-complexed with Mg2+, cis-complexed with Ca2+, and cis-complexed with Mg2+, respectively.
The orientations of the C=O groups were analyzed by the method described in the Supplementary materials (SM). It is assumed here that the adsorption of the metal ions would not change the refractive indexes of the sample/solution interface. According to the fitted χ(2)ppp/χ(2)ssp ratios, the tilt angles of the C=O groups in the phospholipid molecules under different conditions are obtained: θ is 32.8∘ when the phospholipid molecules are not interacting with the metal ions, θ is 62.9∘ when the phospholipid molecules are trans-complexed with Ca2+, θ is 72.8∘ when the phospholipid molecules are cis-complexed with Ca2+, θ is 59.6∘ when the phospholipid molecules are trans-complexed with Mg2+, θ is 64.3∘ when the phospholipid molecules are cis-complexed with Mg2+. It can be seen that the interaction between the metal ions and the phospholipid molecules results in larger tilt angles, which indicates that the C=O groups tend to lie down more at the interface. Such orientation change should come from either the interaction of the metal ions with the C=O groups, or the interaction with the adjacent PO2− groups upon the metal ion binding, as discussed above. It should be noted, there is no obvious peak shift in the SFG spectra after the metal ion binding, indicating that there is no hydration environment change around the C=O groups. As shown in FIG. 5, the ppp spectral line shape are highly distorted due to the interference from the band centered at 1735 cm−1 with the opposite sign. We believe that more likely the orientation change of the C=O groups should be induced by the metal binding to the PO2− groups. Further SFG analysis reveals that the C=O orientation change more for the Ca2+ binding than that for the Mg2+ binding, suggesting that the calcium ions have stronger interaction with the phospholipid head groups.
The SFG spectra in the C−D stretching region are shown in FIG. 6. The vibrational bands centered at ∼2070 cm−1 and ∼2218 cm−1 are the symmetric stretching (ss) and antisymmetric stretching (as) modes of the CD3 groups, respectively. All the C−D stretching signals are more or less the same. We believe that metal ions do not interact with the hydrocarbon chains in the lipid molecules.
To further confirm that the metal ions do not interact with the lipid hydrocarbon chains, the orientation change of the CD3 groups upon the metal ion binding was calculated (see SM for details). All the calculated ratios χas,(2)ssp/χss,(2)ssp for the CD3 groups under different conditions are similar, i.e. −0.33. And the corresponding tilt angle (θ) is about 22∘. So the orientation of the CD3 groups did not change after the metal ion binding for all the cases (trans- or cis- binding with the metal ions). However, as show in FIG. 6 (b, d), the SFG spectral intensities in the CD range decrease when Ca2+ and Mg2+ ions bind to the phospholipid molecules under the trans-ligand binding condition. After the DPPG vesicles are added under the cis-ligand binding condition, the spectral intensities increase distinctively, as shown in FIG. 6(c, e). Since the tilt angles of the CD3 groups do not change, such decrease and increase of the spectral intensities mean that the packed phospholipid alkyl chains become less and more ordered, respectively. Under the cis-ligand binding condition, the exchange of the phospholipid molecules could happen between the DPPG vesicles and the OTS-dDPPG sample, making the lipid alkyl chains more ordered and possibly leading to the hindrance reduction for the cell membrane fusion.
Ulteriorly, the SFG spectra in the OH stretching region are collected for the phospholipid samples binding with Ca2+ and Mg2+, shown in FIG. 7. The trans-complexation for both Ca2+ and Mg2+ leads to the decrease of the SFG spectral intensities, indicating that the phospholipid molecules are dehydrated to make the interfacial water molecules disordered upon interacting with the two metal ions. In the case of cis-complexation, Ca2+ ions lead to further dehydration of the phospholipid molecules, as evidenced by the weakened OH signals, as shown in FIG. 7(a). Such dehydration should promote the membrane fusion. The cis-complexation of Mg2+ with the phospholipid molecules enhances the OH signals, as shown in FIG. 7(b), indicating the existence of a stronger hydration layer at the phospholipid head groups, which should hinder the mutual contact and the further fusion between the dDPPG monolayer and the DPPG vesicles. According to the results of the SFG spectra, a schematic diagram of the interfacial water molecules under different metal binding conditions is shown in FIG. 8. Such spectral results demonstrate that dehydration of the lipid molecules caused by the binding of Ca2+ plays an important role in the cell membrane fusion. Such dehydration capability from Ca2+ must be related to the interaction between Ca2+ and the head PO2− groups in the lipid molecules, as discussed in the previous sections.
The feasibility of using SFG to investigate the metal ion-induced membrane fusion has been demonstrated in this study. Based on the experimental spectral results, we monitored the fusion of the model phospholipid vesicles into the OTS-dDPPG samples induced by Ca2+ and Mg2+ at the molecular level. Judging from the DLS results of the particle size distributions, it can be concluded that Ca2+ can induce the inter-vesicular fusion while Mg2+ cannot. The SFG results indicate that the lipid head PO2− groups interacts with Ca2+ more strongly compared to Mg2+, and the C=O groups and the hydrocarbon alkyl chains do not interact with the two metal ions. Therefore, the binding interaction between the PO2− groups and Ca2+ is likely a pivotal reason for the fusion of the phospholipid membranes. The cis-complexation of Ca2+ with the DPPG phospholipid molecules results in the dehydration of the phospholipid molecular layer; while the cis-complexation of Mg2+ with the DPPG phospholipid molecules could strengthen the hydration of the phospholipid molecular layer. Hence, the membrane fusion can be facilitated by the Ca2+-induced dehydration (likely related to the strong lipid PO2−-Ca2+ interaction), which is consistent with the previous studies on the Ca2+-induced membrane fusion. This study shows that surface/interface sensitive SFG-VS technique can be applied in the field of the membrane biology, elucidating mechanisms of important biological processes related to the cell membrane at the molecular level.
This work was supported by the National Natural Science Foundation of China (No.21773028), and the Fundamental Research Funds for the Central Universities.
[1] |
D. M. Eckert and P. S. Kim, Annu. Rev. Biochem. 70, 777 (2001). doi: 10.1146/annurev.biochem.70.1.777
|
[2] |
S. Hoppins, L. Lackner, and J. Nunnari, Annu. Rev. Biochem. 76, 751 (2001).
|
[3] |
P. L. McNeil and R. A. Steinhardt, Annu. Rev. Cell Dev. Biol. 19, 697 (2003). doi: 10.1146/annurev.cellbio.19.111301.140101
|
[4] |
D. Duelli and Y. Lazebnik, Nat. Rev. Cancer. 7, 968 (2007). doi: 10.1038/nrc2272
|
[5] |
A. Sapir, O. Avinoam, and B. Podbilewicz, Dev. Cell. 14, 11 (2008). doi: 10.1016/j.devcel.2007.12.008
|
[6] |
H. Harashima, Y. Shinohara, and H. Kiwada, Eur. J. Pharm. Sci. 13, 85 (2001). doi: 10.1016/S0928-0987(00)00211-6
|
[7] |
A. A. Gabizon, J. Drug Target. 10, 535 (2002). doi: 10.1080/1061186021000043061
|
[8] |
Y. A. Chen, Nat. Rev. Mol. Cell Biol. 2, 98 (2001). doi: 10.1038/35052017
|
[9] |
J. Diao, Z. Su, Y. Ishitsuka, B. Lu, K. S. Lee, Y. Lai, Y. Shin, and T. Ha, Nat. Commun. 1, 1 (2010).
|
[10] |
B. R. Lentz, Chem. Phys. Lipids. 73, 91 (1994). doi: 10.1016/0009-3084(94)90176-7
|
[11] |
R. Leventis, J. Gagne, N. Fuller, R. Rand, and J. Silvius, Biochemisty 25, 6978 (1986). doi: 10.1021/bi00370a600
|
[12] |
H. R. Marsden, I. Tomatsu, and A. Kros, Chem. Soc. Rev. 40, 1572 (2011). doi: 10.1039/C0CS00115E
|
[13] |
D. K. Struck and D. Hoekstra, Biochemistry 20, 4093 (1981). doi: 10.1021/bi00517a023
|
[14] |
T. Stegmann, P. Schoen, R. Bron, J. Wey, I. Bartoldus, A. Ortiz, J. L. Nieva, and J. Wilschut, Biochem. 32, 11330 (1993). doi: 10.1021/bi00093a009
|
[15] |
D. Hoekstra, T. D. Boer, K. Klappe, and J. Wilschut, Biochemistry 23, 5675 (1984). doi: 10.1021/bi00319a002
|
[16] |
P. L. Yeagle, The Membranes of Cells, 3rd Edn., San Diego/Cambridge/Oxford: Academic Press, (2016).
|
[17] |
W. Wickner and R. Schekman, Nat. Struct. Mol. Biol. 15, 658 (2008). doi: 10.1038/nsmb.1451
|
[18] |
X. D. Zhu, H. Suhr, and Y. R. Shen, Phys. Rev. B 35, 3047 (1987). doi: 10.1103/PhysRevB.35.3047
|
[19] |
X. Zhuang, P. B. Miranda, D. Kim, and Y. R. Shen, Phys. Rev. B 59, 12633 (1999).
|
[20] |
J. Wang, C. Chen, S. M. Buck, and Z. Chen, J. Phys. Chem. B 105, 12118 (2001). doi: 10.1021/jp013161d
|
[21] |
F. Wei, Y. Y. Xu, Y. Guo, S. L. Liu, and H. F. Wang, Chin. J. Chem. Phys. 22, 592 (2009). doi: 10.1088/1674-0068/22/06/592-600
|
[22] |
H. F. Wang, L. Velarde, W. Gan, and L. Fu, Annu. Rev. Phys. Chem. 66, 189 (2015). doi: 10.1146/annurev-physchem-040214-121322
|
[23] |
X. Zhang and Z. Chen, Langmuir 30, 4933 (2014). doi: 10.1021/la500476u
|
[24] |
T. Weidner, N. F. Breen, K. Li, G. P. Drobny, and D. G. Castner, Proc. Nat. Acad. Sci. USA 107, 13288 (2010). doi: 10.1073/pnas.1003832107
|
[25] |
X. Han, Y. Liu, F. G. Wu, J. Jansensky, T. Kim, Z. Wang, C. L. Brooks, J. Wu, C. Xi, C. M. Mello, and Z. Chen, J. Phys. Chem. B. 118, 2904 (2014).
|
[26] |
F. Wei, W. X. Xia, Z. J. Hu, W. H. Li, J. Y. Zhang, and W. Q. Zheng, Chin. J. Chem. Phys. 29, 171 (2016). doi: 10.1063/1674-0068/29/cjcp1601001
|
[27] |
J. Tan, Y. Luo, and S. Ye, Chin. J. Chem. Phys. 30, 671 (2017). doi: 10.1063/1674-0068/30/cjcp1706114
|
[28] |
X. Zhang, J. N. Myers, H. Huang, H. Shobha, Z. Chen, and A. Grill, J. Appl. Phys. 119, 084101 (2016). doi: 10.1063/1.4942442
|
[29] |
B. Ding, J. Jasonsky, Y. Li, and Z. Chen, Acc. Chem. Res. 49, 1149 (2016). doi: 10.1021/acs.accounts.6b00091
|
[30] |
Y. Ma, J. Hou, W. Hao, J. Liu, L. Meng, and Z. Lu, Phys. Chem. Chem. Phys. 20, 17199 (2018). doi: 10.1039/C8CP00975A
|
[31] |
T. Luo, R. Zhang, X. Peng, X. Liu, C. Zhou, X. Yang, and Z. Ren, Surf. Sci. 689, 121459 (2019). doi: 10.1016/j.susc.2019.121459
|
[32] |
S. Wang, W. Sun, S. Guo, X. Liu, and X. Han, Langmuir 37, 4441 (2021). doi: 10.1021/acs.langmuir.0c03551
|
[33] |
R. Zhang, X. Peng, Z. Jiao, T. Luo, C. Zhou, X. Yang, and Z. Ren, J. Chem. Phys. 150, 074702 (2019). doi: 10.1063/1.5066580
|
[34] |
J. Hou, X. Zhang, and Z. Lu, Chem. Phys. 536, 110814 (2020). doi: 10.1016/j.chemphys.2020.110814
|
[35] |
L. Zhang, J. Tan, Q. Pei, and S. Ye, Chin. J. Chem. Phys. 33, 532 (2020). doi: 10.1063/1674-0068/cjcp2006113
|
[36] |
J. Tan, J. Zhang, Y. Luo, and S. Ye, J. Am. Chem. Soc. 141, 1941 (2019). doi: 10.1021/jacs.8b08537
|
[37] |
B. Ding, J. E. Laaser, Y. Liu, P. Wang, M. T. Zanni, and Z. Chen, J. Phys. Chem. B 117, 14625b (2013). doi: 10.1021/jp408064b
|
[38] |
J. Tan, C. Li, J. Zhang, and S. Ye, Chin. J. Chem. Phys. 31, 523 (2018). doi: 10.1063/1674-0068/31/cjcp1805128
|
[39] |
L. L. Olenick, J. M. Troiano, N. Smolentsev, P. E. Ohno, S. Roke, and F. M. Geiger, J. Phys. Chem. B 122, 5049 (2018). doi: 10.1021/acs.jpcb.8b00309
|
[40] |
M. Dogangun, P. E. Ohno, D. Liang, A. C. McGeachy, A. G. Bé, N. Dalchand, T. Li, Q. Cui, and F. M. Geiger, J. Phys. Chem. B 122, 4870 (2018). doi: 10.1021/acs.jpcb.8b02138
|
[41] |
X. Chen, J. Wang, A. P. Boughton, C. B. Kristalyn, and Z. Chen, J. Am. Chem. Soc. 129, 1420 (2007). doi: 10.1021/ja067446l
|
[42] |
X. Chen and Z. Chen, Biochim. Biophys. Acta-Biomembr. 1758, 1257 (2006). doi: 10.1016/j.bbamem.2006.01.017
|
[43] |
X. Chen, A. P. Boughton, J. J. G. Tesmer, and Z. Chen, J. Am. Chem. Soc. 129, 12658 (2007). doi: 10.1021/ja075542w
|
[44] |
P. Yang, F. G. Wu, and Z. Chen, J. Phys. Chem. C 117, 17039 (2013). doi: 10.1021/jp4047215
|
[45] |
B. Li, X. Lu, X. Han, F. G. Wu, J. N. Myers, and Z. Chen, J. Phys. Chem. C 118, 28631 (2014). doi: 10.1021/jp509272k
|
[46] |
G. Ma and H. C. Allen, Langmuir 22, 5341 (2006). doi: 10.1021/la0535227
|
[47] |
X. Chen and H. C. Allen, J. Phys. Chem. A 113, 12655 (2009).
|
[48] |
P. Yang, K. T. Homan, Y. Li, O. Cruz-Rodriguez, J. J. G. Tesmer, and Z. Chen, Biochemistry 55, 2841 (2016). doi: 10.1021/acs.biochem.6b00354
|
[49] |
A. P. Boughton, P. Yang, V. M. Tesmer, B. Ding, J. J. G. Tesmer, and Z. Chen, Proc. Nat. Acad. Sci. USA 108, 667 (2011).
|
[50] |
B. Li, X. Li, Y. H. Ma, X. Han, F. Wu, Z. Guo, Z. Chen, and X. Lu, Langmuir 32, 7086 (2016). doi: 10.1021/acs.langmuir.6b01944
|
[51] |
J. Zhang, W. Yang, J. Tan, and S. Ye, Phys. Chem. Chem. Phys. 20, 5657 (2018). doi: 10.1039/C7CP07389E
|
[52] |
L. B. Dreier, M. Bonn, and E. H. G. Backus, J. Phys. Chem. B 123, 1085 (2019).
|
[53] |
N. N. Casillas-Ituarte, X. Chen, H. Castada, and H. C. Allen, J. Phys. Chem. B 114, 9485 (2010). doi: 10.1021/jp1022357
|
[54] |
X. Chen, W. Hua, Z. Huang, and H. C. Allen, J. Am. Chem. Soc. 132, 11336 (2010). doi: 10.1021/ja1048237
|
[55] |
Y. H. Ma, B. Li, J. Yang, X. Han, Z. Chen, and X. Lu, J. Phys. Chem. C 123, 17899 (2019). doi: 10.1021/acs.jpcc.9b04557
|
[56] |
V. Volkov and M. Bonn, J. Phys. Chem. B 117, 15527 (2013). doi: 10.1021/jp405852r
|
[57] |
W. Wang, J. Tan, and S. Ye, J. Phys. Chem. B 124, 5169 (2020). doi: 10.1021/acs.jpcb.0c02464
|
[58] |
D. Papahadjopoulos, S. Nir, and N. t. Düzgnes, J. Bioenerg. Biomembr. 22, 157 (1990). doi: 10.1007/BF00762944
|
[59] |
R. Leventis, J. Gagne, N. Fuller, R. Rand, and J. Silvius, Biochemistry 25, 6978 (1986). doi: 10.1021/bi00370a600
|
[60] |